Research Highlights 2006
![]() |
![]() |
![]() |
![]() |
TerraneChron®
TerraneChron® is GEMOC’s unique methodology for terrane evaluation. During 2006 industry continued extensive use of TerraneChron® as a cost-effective tool for mapping crustal history on different scales.
Reworking the Archean Gawler Craton, South Australia: U-Pb and Hf-isotopes in detrital zircon
The TerraneChron® methodology was applied to zircons in drainage samples collected from 24 defined catchments across the Gawler Craton, South Australia. The results define the relative contribution of juvenile sources and recycled crust to the continental crust through time and constrain the role of the mantle during the Proterozoic rejuvenation of the Archean craton.
Figure 1. Hf-isotope composition vs age for detrital zircons from the Gawler Craton. Most grains in the 1500-2000 Ma range fall below the CHUR line, indicating derivation largely from older crust (2.5-3.2 Ga). Dashed lines show the evolution of average continental crust of different ages.
Widespread Hf model ages (measured by in situ LAM-MC-ICPMS) of about 3.5 Ga, and the presence of inherited zircon grains with comparable U-Pb ages (3.2 - 3.5 Ga), show that Archean crust as old as ca 3.5 Ga exists in the Gawler Craton. This component may now reside largely in the lower crust, where it has provided a source of crustal magmas throughout the Proterozoic rejuvenation of the craton (Fig. 1).
The Hf isotope data show that juvenile mantle-derived material represents a minor contribution to crustal generation relative to the reworking of older crust. Three periods of juvenile input can be recognised at ca 2540 Ma, 1850 Ma and 1595 Ma (Fig. 2,3). However, only a minor proportion of the zircons formed during these time intervals have strongly juvenile signatures (εHf ≥ 4), and most magmatic rocks represent mixtures of remelted crustal material with mantle-derived material input.
Figure 2. Event Signature curves for Australian tectonic blocks. The Gawler Craton evolved differently from the other three terrains after ca 1900 Ma; it lacks the large juvenile inputs associated with ca 1.7 Ga mineralisation events in the Broken Hill and Mt Isa blocks.
“Event Signature” curves (Fig. 2) allow the visual synthesis of terrane evolution, and a useful way to compare the evolution of different crustal domains; they provide a new tool to advance existing plate tectonic models for the evolution of the Australian continent. Comparison of the Event Signature of the Gawler Craton with those from the Mount Isa Block and Georgetown Inlier shows some similarities in Late Archean-Early Proterozoic time, but makes it clear that the Gawler Craton evolved independently from these terrains, and from the Broken Hill Block, between ca 1800 and 1500 Ma.
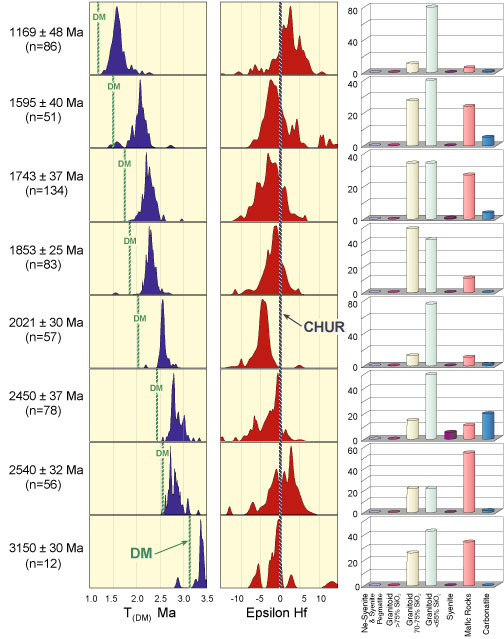
Figure 3. Model ages, epsilon Hf values and modelled rock types for the Gawler Craton in different time slices. Time slices with more mafic rocks show generally higher epsilon values, consistent with more mantle-derived magmatism.
Contacts: Elena Belousova, Bill Griffin
Funded by: ARC, PIRSA, Macquarie University
Heavy Rock(s) in Tamworth
Modelling of detailed gravity data can reveal the subsurface structure of fold belts and their associated faults. For much of the period 400 200 Ma, the southern New England Fold Belt (SNEFB) was a convergent plate margin at the eastern edge of the Gondwana continent with a west-dipping subduction zone. The SNEFB (Fig. 1) consists of the Tamworth Belt in the west and the Tablelands Complex in the east. The SNEFB has been thrust westward over the Permian-Triassic Sydney-Gunnedah Basin, along the Mooki Fault. The Peel Fault, which trends north-northwest, separates the less deformed rocks of the Tamworth Belt from the more deformed rocks of the Tablelands Complex.
Figure 1. Generalised structural units of the southern New England Fold Belt and the Gunnedah Basin. Also shown are locations of the five gravity profiles acquired for this study and the GA seismic line BMR91-G01.
The Bouguer anomaly map of the SNEFB (Fig. 2) has two meridional linear gravity highs: the Namoi Gravity High (NGH) on the east, and the Meandarra Gravity Ridge (MGR) on the west. The NGH lies over the Tamworth Belt along its entire length and lies east of the Mooki Fault in the south and the Kelvin Fault in the north. The eastern edge of the NGH coincides roughly with the Peel Fault. To the west, the MGR lies within the Gunnedah Basin, which otherwise generally shows a relative gravity low. These gravity highs are distinct features and understanding what is causing them should help us to understand the upper crustal structure of the SNEFB.
Five ENE-trending high-resolution gravity traverses (450 readings) were conducted across the belt, adjacent parts of the Gunnedah Basin and the Tablelands complex. These data were modelled together with density determinations of surface and drillcore samples. The data were first modeled using the geometry derived from the interpretation of GA’s BMR91-G01 seismic line.
This modelling (Fig. 3) indicated that the gravity anomalies correlate with the densities of the exposed rock units. The Namoi Gravity High over the Tamworth Belt is produced by the high density of the rocks in this belt. These high densities reflect the mafic volcanic source of the older sedimentary rocks in the Tamworth Belt, the burial metamorphism of the pre-Permian units and the presence of some mafic volcanic units. Modelling shows that the Woolomin Association, present immediately east of the Peel Fault and constituting the most western part of the Tablelands Complex, also has a relatively high density, which also contributes to the NGH. The Tamworth Belt can be best modelled with a configuration in which the Tablelands Complex has been thrust over the Tamworth Belt along the Peel Fault, which dips steeply to the east to a depth of more than 10 km. Plutons near the Peel Fault (eg Moonbi) are also clearly delineated by the data. The Tamworth Belt is thrust westward over the Sydney-Gunnedah Basin for 15-30 km on the Mooki Fault that has a shallow dip (~25°) to the east and is consistent with the new gravity data.
Figure 2. Bouguer gravity anomaly image covering the southern New England Fold Belt and the Gunnedah Basin, with location of the gravity profiles surveyed in this study. Unit: µm/s2. Red lines indicate the Peel and Mooki Faults. Also shown is the seismic line BMR91-G01.
The Meandarra Gravity Ridge within the Gunnedah Basin was modelled as a high-density volcanic rock unit with a density contrast of 0.25 tm-3 relative to the underlying rocks of the Lachlan Fold Belt. The modelled unit has a steep western margin, a gently tapering eastern margin and a thickness ranging from 4.5 - 6 km. These volcanic rocks are assumed to be Early Permian in age; they may be the western extension of the Permian Werrie Basalts that outcrop on the western edge of the Tamworth Belt, and that may have formed within an extensional basin.
Figure 3. 2.5D gravity model along the Tamworth Profile (cross-section view) shows a good fit between the observed data and calculated profile. The Peel Fault was modelled as a steep east-dipping fault extending to mid crustal levels. Crosses represent observed data and solid line is the calculated anomaly, V/H=1.9. Density in tm-3. Dashed straight line is the regional gradient.
Contacts: Mark Lackie, Dick Flood, Bin Guo
Funded by: Macquarie University Postgraduate Research Fund
How did the Earth form?
From observations of newly-forming stars it seems that our solar system developed from a flattened disk of dust and gas around the young sun 4567 million years ago. The mechanisms of growth of particles of dust into asteroidal (20 km diameter) bodies are not well understood, but once this size had been reached, gravitational attraction would have ensured growth to planetary-size bodies. Understanding of these processes of planetary growth and their timing can be obtained by studying the chemical compositions of the planets and asteroids and the characteristic isotopic signatures for some key elements.
Figure 1. Abundance in silicate Earth versus temperature of 50% condensation.
Amongst the thousands of meteorites in museum collections there are about a dozen that appear to represent primitive protoplanetary material. These “carbonaceous chondrites” come from the asteroid belt between Mars and Jupiter and have compositions which closely mimic that of the Sun. They also have, for elements that condense at high temperatures from a gas of solar composition, striking affinities with the Earth, Mars and the Moon. Figure 1 shows ratios of element abundances in the silicate part of the Earth (mantle+crust) to those in carbonaceous chondrites, plotted against the temperature at which 50% of the element of interest would condense from a solar gas. Refractory elements, which condense at high temperatures, are divided into 3 groups, “lithophile”, “siderophile” and “highly siderophile”. Lithophile elements such as Ca, Ti, Hf, Al and the Rare Earths are those elements which did not enter the Earth’s iron-rich metallic core. They are present in the same relative abundances in the silicate Earth as in the meteorites, Mars and the Moon. The siderophile elements (Fe, Ni, Mo, W etc) are all depleted in the silicate part of the Earth because they have partitioned into the core, while the highly siderophile elements (Au, Pt etc) are >99% partitioned into the core. The Earth is also depleted in volatile elements (those with low condensation temperatures) because of late volatile loss.
Figure 2. Deep magma ocean model of core segregation.
Asteroids, many of which have metallic cores like the Earth, are known to have formed within a few million years (some as soon as 1 Ma) after the beginning of the solar system. This result was obtained using the radioactive decay of 182Hf (a short-lived isotope present when the solar system formed) to 182W. Hafnium is lithophile while tungsten (W) is siderophile (Fig. 1). Therefore as core formed on any body, most of the tungsten was extracted to the core, leaving the silicate part with a very high Hf/W ratio. The build-up of 182W during the first 50 Ma of solar system history (by which time all 182Hf had decayed) then dates the time at which Hf and W were separated by core formation. Application of this method to Mars gives a time about 10 Ma after the beginning of the solar system and the Earth about 30 Ma. This then enables us to estimate the timescales of formation of asteroids (1-4 Ma), a planet 10% of Earth-size (Mars) and Earth itself.
Research at Macquarie is concentrating on studying the conditions on Earth as the metal core formed. Earth grew by initially sweeping up lots of smaller bodies, then progressively fewer larger and larger bodies, culminating in the impact of a Mars-sized body which generated the moon about 40 Ma after the beginning of the solar system. Our experimental work is aimed at finding the pressures and temperatures under which the siderophile element contents of the silicate Earth (Fig. 1) match those observed. We find that the metal was extracted at high pressures, probably at the base of a deep (>400 km) ocean of molten silicate (Fig. 2). This magma ocean was kept molten by the high energies of impacting bodies. We also find that the Earth appears to have become more oxidised as it grew. It is likely that the earliest atmosphere (during early growth of the Earth) was hydrogen-rich, solar-like in composition, and strongly reducing. However, this was blown away (together with many volatile elements; Fig. 1) by large impacts later in accretion, leaving the Earth to oxidise by a combination of internal and external processes.
Contact: Bernie Wood
Funded by: ARC (Federation Fellowship, Discovery Project)
Flushing the mantle: Harzburgite to lherzolite in the Lherz Massif (France)
Differentiation of the earth’s mantle occurs mainly through partial melting and extraction of basaltic melts. Among the mantle rocks occurring at the Earth’s surface, lherzolites are widely regarded as samples of the pristine mantle, from which no (or only small amounts of) melts have been extracted. In contrast, harzburgites are seen as refractory mantle residues left after extensive partial melting.
The Lherz Massif (Pyrenees, France; picture above) is the type locality of lherzolite. The massif is predominantly composed of layered spinel lherzolites; in the upper part of the massif the lherzolites enclose bodies of highly refractory spinel harzburgite metres to tens of metres in size (Fig. 1).
Detailed structural mapping shows that all harzburgites, even small bodies, have a constant foliation throughout the massif. In contrast, the foliation in the lherzolites is variable and generally oblique to the harzburgite foliation. This suggests that the lherzolites are secondary rocks, and that the harzburgites are remnants of a highly refractory mantle protolith, largely replaced by the lherzolites through a process of melt percolation.
Crystallographic orientations were measured by indexation of electron back scattered diffraction (EBSD) patterns at the Géosciences Montpellier laboratory. Analysis of microstructures and crystal-preferred orientations suggests that the harzburgites deformed by dislocation creep with activation of the high temperature, low stress (010) [100] slip system. Refertilised lherzolites display a much weaker alignment of the a axis of olivine (Fig. 2).
Figure 1. Harzburgite-lherzolite contacts are sharp and steep, and emphasised by a cm-scale websteritic layering.
These features suggest that melt percolation and melt-rock reaction started at static conditions, and produced changes in modal composition, grain growth and a weakening of the olivine fabric.
Variations of major, minor and trace elements across the harzburgite-lherzolite contacts indicate that the lherzolites were formed by a near-solidus refertilisation reaction involving crystallisation of pyroxene and spinel, and dissolution of olivine. These processes produce chemical trends in the relationships between mineral and whole-rock chemistry that are different in detail from partial-melting trends (Fig. 3).
Figure 2. Illustration of the high temperature deformation in Lherz harzburgites and the weakening of crystallographic fabrics in refertilised lherzolites.
The combination of detailed fieldwork, petrophysical analysis and geochemical modelling reveals that the type lherzolites of the Lherz massif represent refertilised, rather than pristine, mantle. The refertilisation process has involved the interaction of refractory lithospheric mantle with upwelling asthenospheric partial melts. This study highlights the fact that melt transport and melt-rock reaction play a key role in modifying the composition of the lithospheric mantle, and that models of lithospheric mantle composition based on such lherzolites must be reconsidered.
Figure 3. Modelling of partial melting versus refertilisation reaction. Orange squares, harzburgites; green circles, lherzolites.
Contacts: V. Le Roux, J.-L. Bodinier, S.Y. O’Reilly
Funded by: Bourse MENRT, INSU
Mantle “Botox” and the tale of the primitive upper mantle
One of the recurrent questions in planetary science is what the Earth is made of; what were the “building blocks” of our planet? Of special interest is the nature of the ‘late veneer’, a final meteoritic bombardment that may have been the main carrier of water and possibly life-seeds to Earth. However, the nature of this late component remains elusive, and constraints from various geochemical systems seem at first glance to be contradictory. In that respect, the siderophile elements may be one of the most promising sources of first-hand constraints on the nature of the late accreting material. The broadly chondritic relative abundances of highly siderophile elements (HSE) in the Earth’s mantle are assumed to reflect the addition of chondritic material to the mantle after core formation. The HSE composition of the Primitive Upper Mantle (PUM) is thus an important goal of modern geochemistry. Current estimates of PUM for siderophile elements (PGE and Os isotopes) are based largely on two suites of mantle samples: peridotite xenoliths from Kilbourne Hole maar in Texas, and the Lherz orogenic peridotite massif in the Pyrenees (Le Roux et al., Earth and Planetary Science Letters, in press; see "Flushing the Mantle..."). The Os isotopic composition of the Earth’s Primitive Upper Mantle (187Os/188Os =0.1296 ± 0.0008) is significantly higher than the ratio measured in carbonaceous chondrites (CC; 187Os/188Os = 0.1262 ± 0.0006). Taken at face value, this estimate thus rules out CC as the source of the ‘late veneer’ and suggests that ordinary chondrites (OC) formed the bulk of the ‘late veneer’. However, carbonaceous chondrites are the only water-bearing chondrites and have a Deuterium to Hydrogen ratio (D/H) similar to the Earth’s ocean (≈ 150 x10-6).
Figure 1. Os-isotope compositions of sulfide grains in samples of the primary harzburgite, the refertilised lherzolite and transitional rocks in the Lherz massif. Bars show the 187Os/188Os corresponding to the “aluminochron age” and the “PUM” estimate derived from these rocks; both are spurious.
These sorts of inconsistencies have led to proposals that the Earth was made of an unsampled type of material dubbed “Earth-chondrite” or “Earth achondrite”. However, before calling upon the existence of a never-sampled material, we need to examine the robustness and significance of the PUM estimate. Indeed, the same mantle-sample suites also yield a Pd/Ir (≈ 2) much higher than any type of chondrite. Since Os, Ir, Pd and Re are all HSE and thus should behave similarly, this inconsistency raises suspicion about the nature of the data.
The Lherz Orogenic massif is the type area of lherzolite (usually assumed to represent the dominant rock type of the upper mantle) and has always been a key area for studying mantle composition and processes. However as demonstrated in the previous highlight (see "Flushing the Mantle...") structural, petrographic and geochemical features clearly indicate that the lherzolite suite was developed at the expense of the more refractory harzburgite via a refertilisation reaction involving precipitation of pyroxene (±spinel) and sulfide at the expense of olivine and infiltrated melt.
In situ measurement of the Os composition of rare sulfides in the harzburgites yields a constant unradiogenic Os composition indicating a TRD age ≈ 2.4 Ga (Fig. 1). In contrast, the numerous sulfides in the lherzolite show a large spread of Os composition (0.11 ≤ 187Os/188Os ≤ 0.18). However, the unradiogenic sulfides characteristic of the harzburgite are common in the lherzolite, while extremely radiogenic Os is characteristic of Lherz’s pyroxenite suite. The whole-rock composition of individual lherzolite samples are intermediate between these two end members, depending on the proportion of each sulfide population (Fig. 2). This indicates that the lherzolites are in fact old, refractory harzburgites which were re-fertilised by fluids that carried radiogenic Os. This conclusion is consistent with the structural, petrographic, petrophysical and geochemical evidence presented by Le Roux et al. (in press).
Our previous work has demonstrated that the abundances of sulfides and HSE in several of the xenolith suites used to derive the Os-isotope composition of PUM have been heavily altered by metasomatic events. Together with the Lherz study, the results cast strong doubt on the robustness and significance of the currently accepted Os-isotope composition of the PUM, its bulk composition and the long-term evolution of the mantle. It appears that the “PUM” in fact represents refertilised/metasomatised, old, depleted upper mantle; it lost its “primitive” character a long time ago.
Recognition that the currently accepted model composition for the PUM is not meaningful can help to resolve some of the contradictions noted above. A better estimate of the PUM and the original bulk composition of the Earth will come through detailed studies of the budgets of siderophile and chalcophile elements in a dynamic Earth. This will need a better understanding of metasomatic processes, detailed studies of key terrestrial and meteoritic sample suites and integration with experimental studies (see "How did the Earth form?").
Figure 2. Al2O3 vs. 187Os/188Os for whole-rock samples as used to define the PUM Os composition and the Os isotopic variability of the sulfides in each sample, Red triangles are the websterite and other pyroxenites in Lherz.
Contacts: Olivier Alard, Sue O'Reilly, Bill Griffin
Funded by: ARC-DP/APD
Building an oceanic plateau: The Kerguelen Archipelago
The kerguelen archipelago, located in the southern Indian Ocean (49°S and 69°E), is the emergent part of the northern Kerguelen oceanic plateau (Fig. 1).
Figure 1. Simplified geological map of the Kerguelen Archipelago, after Delpech (GEMOC Research Highlights 2003).
The magmatic edifice of the Kerguelen Islands was produced over about the last 40 Ma. When the volcanic activity associated with the Kerguelen Plume started, the Kerguelen area was situated near or on the South East Indian Ridge (SEIR). The SEIR then moved away to the NE, leaving the Kerguelen Plateau in its present intraplate setting. The early magmatism was dominantly of tholeiitic-transitional affinity and became progressively alkaline to highly alkaline over time. Therefore the Kerguelen Archipelago has a complex history, superposing in time and space two types of hotspot activity. Between ~40 and ~26 Ma, the volcanism was similar to Iceland (interaction between a hotspot and a ridge); since 26 Ma, it was more similar to the Hawaiian (intraplate) hotspot type.
The Kerguelen Archipelago is made up mainly of flood basalt (80%), plutonic rocks (5%), and dykes with ultramafic and mafic xenoliths.
Over the last fifteen years, petrologic, geochemical and isotopic studies have mainly focused on the flood basalts and ultramafic xenoliths brought up from the upper mantle by alkaline basalts. These studies have characterised (i) the petrologic and geochemical heterogeneity of the mantle, (ii) the nature of the magmas and fluids circulating through the lithosphere, (iii) the different mantle sources. Geochemical studies coupled with geophysical studies have shown that the oceanic lithosphere was thickened by underplating of mafic magmas under the Kerguelen archipelago.
Gabbroic rocks (gabbros and meta-gabbro) are found at different structural levels in the oceanic lithosphere (crust, mantle/crust boundary and upper mantle), but they have been studied very little. The characterisation of these intrusive rocks (gabbroic and hypovolcanic) and related cumulate ultramafic-mafic xenoliths will help to understand the different stages of lithospheric differentiation in the context of a thickened oceanic crust. These rocks are the key to understanding how magmas evolved from their source to the surface. In a more general context, this work will also help to better constrain the formation of Large Igneous Provinces (LIP).
Figure 2. Gabbroic sill, Val Travers, Kerguelen Island (DyLioKer campaign 2006). Photo: Damien Guillaume.
The gabbros and xenoliths for the initial work came from the St-Etienne University collection (France). Further sampling was done in December 2006 during the DyLioKer campaign supported by IPEV (Institut Paul Emile Victor). The gabbros and other plutonic rocks are exposed in massifs, sills (Fig. 2) and stratified or ring complexes. Some have also been found as xenoliths in alkali basalts.
Both in situ and whole-rock techniques have been used to characterise the gabbros in terms of major and trace elements (microprobe, XRF, LA-MC-ICPMS). The preliminary studies show that several types of gabbroic and intrusive rocks can be distinguished on the basis of their rare earth element (REE) composition. The REE patterns of the clinopyroxenes from different localities (Fig. 2) vary between two end-members. A tholeiitic-transitional pattern is characterised by low light REE contents (LREE, La to Sm) compared with the heavy REE contents (HREE, from Tb to Lu). A second highly alkaline pattern is characterised by high LREE/HREE. Compositions intermediate between these end-members are also observed.
The preliminary results on the gabbroic rocks are consistent with previous studies that indicate two types of magmatic trends, from tholeiitic-transitional to alkaline, and interaction between them. Isotopic studies of the samples will help to better constrain the sources of the different gabbroic rocks, and U-Pb dating of zircon will also put better constraints on the evolution of the different sources with time.
Figure 3. REE patterns measured in the cores and rims of clinopyroxenes in gabbro sampled in different localities on the Kerguelen Archipelago (red lines = alkaline composition, blue lines = tholeiitic-transitional composition, green lines = intermediate compositions).
Contacts: June Chevet, Sue O’Reilly
Funded by: iMURS, ARC-DP
Using kimberlites as drill holes: Crustal evolution beneath the Kundelungu Plateau (D.R. Congo)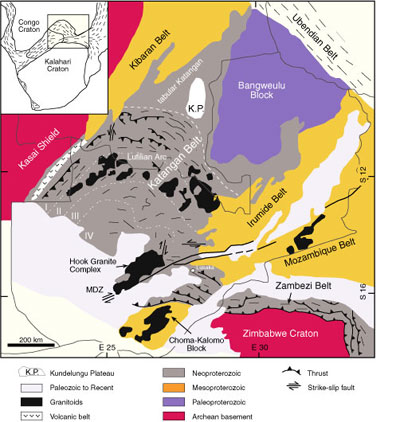
The kundelungu plateau is located in the SE corner of the Democratic Republic of the Congo. Twenty-four kimberlitic pipes are known in the area; recent dating of perovskites at GEMOC shows that they intruded 33±3 Ma ago. The Plateau is formed of rocks belonging to the Neoproterozoic Katangan belt, which is surrounded by the Archean Congo Craton, the Paleoproterozoic Bangweulu Block, the Paleo- to Mesoproterozoic Irumide Belt, the Mesoproterozoic Kibaran Belt and Choma Kalomo Block. The Archean Zimbabwe Craton lies to the south (Fig. 1). The Gungwania and Talala kimberlitic pipes on this plateau have been used as drillholes, to obtain crustal zircons for a study of crustal evolution in the region and to constrain the age of the basement and the sedimentary provenance of the Katangan Supergroup. Zircons were separated from heavy mineral concentrates collected in streams that crosscut these pipes (Fig. 2).
Figure 1. Simplified geological map of the Katangan belt and surrounding basements (modified after Binda and Porada, 1995), showing the location of the Kundelungu Plateau (K.P.).
229 zircons were analysed for U-Pb ages (LAM-ICPMS) and Hf isotope (LAM-MC-ICPMS) compositions. The analyses show that the oldest juvenile crust in the region is approximately 3.4 Ga old, and underwent varying degrees of recycling during Neoarchean and Paleoproterozoic times. The major Paleoproterozoic event is characterised by little production of juvenile crust; any juvenile input was essentially mafic in composition (Fig. 3). Important bimodal magmatism affected the region in the Mesoproterozoic (1 Ga) and Neoproterozoic (700-568 Ma). These events correspond to the continental extension and rifting related to the break-up of the Rodinia supercontinent (~1 Ga), the opening of the Mwashia basin (~700 Ma) and the Lufilian orogeny. The generation of Mesoproterozoic and Neoproterozoic crust involved recycling of Paleoproterozoic crust but these periods are also characterised by an important input of juvenile material (Fig. 3). The existence of a high-176Lu/177Hf (0.059) Archean crust (eg garnet-rich rocks) in the region is suggested by the presence of zircons that lie above the Depleted Mantle line, on a line that projects back to 3.4 Ga (Fig. 3).
Figure 2. Jacques Batumike collecting heavy mineral concentrates on the Talala River (near Talala pipe, Kundelungu Plateau).
The zircons in these samples may come both from the basement and from the Katangan Supergroup sediments. Morphological examination of the zircon grains in different age groups shows that the Paleoproterozoic population has the highest proportion of euhedral zircons, which implies that the basement rocks may be of this age. Most Archean zircons are rounded, indicating long transport. This does not support the presence of an Archean crust beneath the region. However, the Hf-isotope data may indicate that an Archean crust existed at depth at least during Paleoproterozoic time (Fig. 3).
The sediments constituting the Katangan Supergroup were derived from most of the older rocks in the region including the Archean Congo Craton, the Paleoproterozoic Ubendian Belt (Bangweulu Block), the Paleo- to Mesoproterozoic Irumide Belt, the Mesoproterozoic Kibaran Belt and the Choma-Kalomo Block, with a possible minor contribution from the Zimbabwe Craton far south of the Katangan Basin. The absence of any zircons with ages < 560 Ma suggests that the Biano Subgroup sediments were deposited during the Lufilian orogeny.
Figure 3.
A. Age, Hf-isotope and rock type data for each sample and calculated crustal ages. CHUR: Chondritic Uniform Reservoir. Dashed lines show evolution of crustal volumes with 176Lu/177Hf = 0.015, corresponding to the average continental crust.
B. Event signature curve. Source crustal residence time is the time from the separation from the Depleted Mantle to the crystallisation of the zircon. In this plot, an upward trend with decreasing age indicates juvenile input, while a downward trend implies reworking of older crust. The most pronounced juvenile input occurred during the Neoproterozoic events.
Contacts: Jacques Batumike, Sue O’Reilly, Bill Griffin
Funded by: iMURS, IPRS, ARC-DP
The ghosts of lithospheres past: Imaging a changing mantle in Southern Africa
Geologists and geophysicists view the subcontinental lithospheric mantle (SCLM) in different ways at vastly different scales. Geologists can characterise the chemistry and physical state of relatively small volumes of the lithosphere in detail, usually at the scale of an outcrop or the xenoliths sampled in a volcanic eruption. These parameters also contain a time component since the material being studied has been removed from the mantle at a specific point in time. Geophysicists must sample the SCLM in the present using methods that are sensitive to both chemical and physical properties, but at scales many orders of magnitude larger. They then must invert their data to extract information on thermal and chemical properties of the SCLM. Neither of these approaches can show detailed, large-scale maps of the properties of the lithosphere through time, due to the paucity of available material to study (geology) and limitations on sensitivity in time and space (geophysics).
Figure 1. The study area: red triangles, older Group 2 kimberlites; green squares, younger Group 1 kimberlites. The Brakbos Fault is commonly taken as the boundary of the Kaapvaal Craton.
Southern Africa presents a unique opportunity to rectify this; kimberlite intrusions are both spatially and temporally widespread, and provide an abundance of xenolithic material sampled in different time slices. In the southwest corner of the Kaapvaal Craton a cluster of kimberlites from two different intrusive events provides material from the same volume of mantle and allows direct comparison of SCLM composition and structure across the craton margin in two time slices (Group II kimberlites at 115-130 Ma, Group I kimberlites at 85-105 Ma) (Fig. 1). Using methods developed at GEMOC and newly available data from collaborative work with De Beers Exploration we have been able to make more detailed images of the lithosphere than was previously possible with either geologic or geophysical methods, using garnet xenocrysts extracted from the kimberlites.
Geotherms can be derived from the Ni and Cr content of the garnets. Combining these data with the distribution of garnets depleted in Y defines a “chemical” lithosphere-asthenosphere boundary. This coincides with the depth where thermobarometry on xenoliths from other localities indicates a kink in the geotherm, and there is strong enrichment in incompatible trace elements (Fig. 2). The garnet thermobarometry provides a framework on which we can overlay other garnet chemical information, such as Ti content, and also information about the calculated “whole-rock” Al content or the calculated Mg# (Mg/(Mg+Fe)) of coexisting olivine.
We have projected these data onto the cross-section indicated in Figure 1 and produced a continuous surface with a gridding algorithm. Figure 3 is the resulting image for the Mg# of coexisting olivine. This parameter is a sensitive indicator of melt depletion (high Mg#) and metasomatic refertilisation (low Mg#). There is marked change in the SCLM between the eruption of the Group 2 kimberlites (130-115 Ma) and the eruption of the Group 1 kimberlites (105-85 Ma), reflecting a thinning and overall re-enrichment of the depleted SCLM in this area. Both images indicate that the depleted cratonic SCLM extends at least 50 km SW of the Brakbos Fault, usually taken as the craton margin.
Figure 2. Derivation of the garnet geotherm for Group 1 kimberlite Pampoenpoort. A kink in the geotherm is placed at the lower limit of Y depletion, in line with observations on peridotite xenoliths.
The Mg# of olivine has particular geophysical significance because seismic velocities are very sensitive to the Mg/Fe of olivine and other upper mantle minerals. By integration of all the chemical and physical data available through garnet chemistry and mineral elasticity data, it is possible to use data such as those in Fig. 3 to calculate models of seismic wave speeds. In areas with appropriate distribution of data both spatially and temporally, we can in essence make historical seismic images of the SCLM and its evolution through time. In some cases this can be done at higher resolutions than is currently possible with geophysical methods. Continuing collaboration with De Beers Exploration will further develop these methods and extend the models across the entire Kaapvaal Craton and its surrounding mobile belts.
Figure 3. Vertical and lateral distribution of Mg# of olivine coexisting with Cr-garnets projected along section A-A’ (Fig. 1) for two time slices.
Contacts: Alan Kobussen, Bill Griffin, Sue O’ReillyFunded by: iMURS, MUECRG (De Beers)
Eclogites in the SCLM: The subduction myth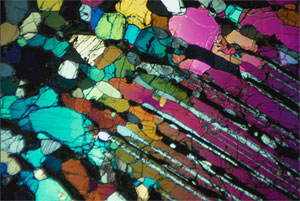
It has become conventional wisdom that eclogite and garnet pyroxenite xenoliths derived from cratonic subcontinental lithospheric mantle (SCLM) represent fragments of subducted ocean floor, implying that the SCLM has grown by a lithosphere-stacking mechanism involving repeated shallow subduction beneath cratons. However, the implied behaviour of these ancient “slabs” is markedly different from what we observe in the modern Earth; seismic-tomography images clearly show slabs descending steeply to at least 660 km depth, rather than layering at shallow depths beneath the continents.
Figure 1. High-temperature clinopyroxenite (crystallised in the lithospheric mantle from mafic magma) exsolving lamellar garnet and orthopyroxene, and recrystallising to produce a websterite.
Xenolith suites in basalts from young terrains (Tectons: eg E. China, E. Australia, western USA, Hawaii) commonly contain garnet pyroxenites that display exsolution microstructures clearly reflecting their origin as high-T cumulates or crystallised melts (Fig. 1). Similar microstructures also occur in cratonic eclogites. The compositional field of Tecton garnet pyroxenites can be expressed by mixing of high-T, high-Al cpx ± opx ± gnt. This compositional field is coincident with that of nearly all cratonic eclogites (reconstructed from mineral compositions); both rock types are distinct in composition from clearly metabasaltic eclogites in HP/UHP metamorphic belts (Fig. 2). Many eclogites also have experienced episodes of metasomatism, making bulk compositions (especially trace element patterns) an unreliable guide to their origin.
Simultaneous solutions of cpx-gnt thermometers with the equations for xenolith-derived geotherms show that rather than being widely distributed in the SCLM as implied by lithosphere-stacking models, eclogites from many cratonic areas are concentrated in layers <20 km thick, co-spatial with a strong signature of metasomatism in the surrounding peridotites (Fig. 3). In many cases this combination of features defines a “lithosphere-asthenosphere boundary” marking the transition from depleted SCLM to more fertile underlying mantle. This pattern strongly suggests that the eclogites reflect the intrusion of asthenosphere-derived melts near compositional/rheological boundaries, causing metasomatism in their peridotite wall-rocks.
Figure 2. Compositions of cratonic eclogite suites, calculated from mineral chemistry assuming that gnt:cpx=1. Yellow shaded field encompasses data from whole-rock compositions for Phanerozoic garnet pyroxenite xenoliths in alkali basalts from SE Australia and Hawaii and pyroxenite dikes from European peridotite massifs.
The strongest argument for a crustal origin for cratonic eclogites is the large spread in δ18O observed in some suites; such fractionation is commonly thought to require a low-T origin. However, Mg isotopes in high-T peridotites show equally large fractionation even within single xenoliths (Pearson et al., 2006); significant isotopic fractionation clearly can take place at T >1000°C. SCLM eclogites commonly host diamonds with low-δ13C carbon; this has been interpreted as biogenic in origin, but this model is not consistent with N-isotope data (Cartigny et al., 1998). The δ13C variation can instead be explained by Rayleigh fractionation during redox reactions (Muroka et al., 2005). In framesites, the covariation of δ13C in diamond and δ18O in cogenetic silicates suggests that similar redox-related fractionation mechanisms are involved (Fig. 4); the O-isotopic signatures thus are not prima facie evidence of a shallow origin for SCLM eclogites. Eu anomalies in cratonic eclogites also have been presented as evidence of the previous presence, or fractionation, of plagioclase. However, similar anomalies are found in peridotitic phases, and may simply reflect redox processes during metasomatism.
Figure 3. Distribution of eclogites in the SCLM beneath the Udachnaya kimberlite, Yakutia.
Some SCLM eclogites carry “crustal” radiogenic-isotope signatures -- but so do many intraplate magmas. These signatures may reflect derivation of parental magmas from deeply subducted crust, rather than the direct emplacement of ocean floor into the SCLM. The cratonic eclogites, like the Tecton pyroxenites, may be telling us about the growth or erosion of the SCLM from below, through magmatic processes, rather than from the side, through shallow subduction.
Figure 4. of diamonds vs δ18O in coexisting silicates.δ18O
Contacts: Bill Griffin, Sue O’Reilly
Funded by: ARC Discovery Project
Ancient mantle in modern plumes: B and Os in the Azores
Figure 1. Cartoon of convecting mantle showing cold down-wellings (subducting slabs) in blue and hot upwellings from the core-mantle boundary (plumes) in orange. Kellogg et al., 1999.
The dynamics of the earth reflect its internal heat but the nature and time scales of mantle convection remain poorly constrained. Over the past decade tomography data have provided spectacular images of seismically fast material, associated with subducting plates. These are interpreted as the cool slabs descending through the warmer mantle, suggesting that the slabs can penetrate the 670 km discontinuity, continue into the deep mantle and pond at the core-mantle boundary. Conversely, a significant component of return flow is associated with mantle plumes; many of these, including the Azores, appear to rise from the core-mantle boundary (Fig. 1). These observations have generated a lot of interest in the possibility that subducted material is entrained in these plumes. These ideas can be independently tested by examining the composition of ocean island basalts (OIB) erupted above plumes. Radiogenic isotopes have long been employed in this search because of their potential to constrain the time scales of recycling. Many OIB have indeed been found to have signatures distinct from those of mid-ocean ridge basalts (MORB) that sample the uppermost mantle.
![]() |
![]() |
Figure 2. Two of the Azores islands: (left) The Caldeira das Sete Cidades on São Miguel and (below) the Island of Pico, dominated by the stratovolcano, Pico Mountain.
However, although such signals undoubtedly reflect the time-integrated effects of fractionated parent-daughter element ratios, the age and extent of this fractionation can rarely be sorted out. Even if this is possible, the parent-daughter fractionation is not restricted to processes occurring near the Earth’s surface and could instead reflect intra-mantle metasomatism. In contrast, the isotopes of light elements, such as O, B and Li, are commonly fractionated by low-temperature processes near the Earth’s surface and significant variations in the stable isotope ratios of MORB and OIB could provide evidence for contributions from recycled material. However, the range of O and B isotope ratios observed in MORB and OIB is rather restricted and observed variations often are attributed to shallow assimilation of altered oceanic crust. Furthermore, stable isotopes cannot be used to constrain the time scales of recycling.
A recent study of basalts from the Azores islands (Fig. 2) has found high Nb/B ratios and a large range in δ11B ratios, which provide compelling evidence for the recycling of materials that have undergone fractionation near the Earth’s surface. Moreover, δ11B is negatively correlated with 187Os/188Os ratios that extend to subchondritic values (Fig. 3). The low 187Os/188Os constrains the age of the high Nb/B, 11B-enriched end-member to be ≥ 2.5 Ga. This is inferred to be melt- and fluid-depleted lithospheric mantle from a subducted oceanic plate; other Azores basalts contain a contribution from
~ 3 Ga enriched basalts (Schaefer et al. 2002, Nature 420, 304). The simplest interpretation is that both components are derived from an Archean oceanic plate that was subducted into the deep mantle, where it was stored until thermal buoyancy caused it to rise beneath the Azores islands.
Contact: Simon Turner
Funded by: ARC Federation Fellowship
Figure 3. Variation of Os isotopes with other geochemical indices in the Azores (see text for discussion).
The tenacity of cratons: a low stress home environment
The cratonic regions of the earth’s continental crust have avoided deformation for billions of years. This by itself is not remarkable -- the surfaces of the Moon and Mars have avoided deformation for far longer. However, considering that the oceanic crust, representing nearly 70% of the Earth’s surface, is recycled on a timescale of 100 Myr, and most continental regions are reworked over similar periods, then the survival of cratons becomes an enduring geophysical mystery.
Cratons themselves have a number of physical characteristics that may account for their strength. The crust of cratons overlies a thick, buoyant chemical lithosphere. Cratonic lithosphere exhibits extreme degrees of melt depletion, accounting for its buoyancy with respect to the mantle. The depletion also has led to very low water contents, imparting a potential 100-fold increase in viscosity compared to the asthenosphere. On top of that, cratons are cold, and their deep root regions are expected to be even more viscous and resilient as a result.
Figure 1. The fate of buoyant lithosphere without any instrinsic strength in a strongly convecting Archean mantle. Despite its convective buoyancy, the lithosphere rifts in response to the regional stress regime and is partially recycled into the mantle (O'Neill et al. 2007).
The problem arises when one tries to model cratons with these properties in a convecting mantle - they just aren’t extreme enough. Buoyancy itself cannot impart strength and give rise to cratonic stability - buoyant continents either spread out under their own gravitational instability, get entrained bit-by-bit by downwelling mantle and subducting slabs, or at the least are grossly deformed by their dynamic environment. Previous mantle simulations have shown that viscosity can impart stability, but the high viscosities required are far beyond reasonable bounds for cratonic mantle. For plausible viscosity contrasts, the models tell us that cratons should have been heavily deformed, reworked, and even wholesale recycled under Archean mantle conditions.
And yet the cratons are still here. What factors give rise to their remarkable tenacity? Geodynamic simulations performed at GEMOC have explored these factors in detail, and have shed light on the survival of these economically important terranes. While most previous simulations have focused on the properties of cratons themselves, few have adequately addressed their dynamic environment - the convecting mantle.
Convection in the Earth differs from simple “box” convection simulations in some important ways. Firstly, strongly temperature-dependent viscosity, with brittle/plastic failure and strain-rate weakening, is needed to simulate plates - a condition met in some previous studies. Secondly, the Earth’s mantle displays a strong variation in viscosity with depth, but this effect has not been incorporated in previous craton simulations. This strongly affects the dynamics of the mantle, and the surface stress regime. Thirdly, an endothermic phase change at 670 km hinders slab penetration into the lower mantle, strongly affecting mantle dynamics and its thermal evolution. It also gives rise to a curious phenomenon known as “mantle avalanches”, where material piled up at 670 km periodically breaks through into the lower mantle, instigating a corresponding injection of hot material into the upper mantle. These violent episodes have been associated with crustal production in the past, but represent a highly destructive surface regime for existing cratons.
The main results of the new modelling show that realistic mantle viscosity structures cushion cratons from mantle dynamics; a low-viscosity asthenosphere partly decouples the surface plates from the mantle beneath, and a viscous lower mantle mitigates the stress extremes experienced by cratons during mantle avalanche events. Under the hotter mantle conditions of the past, however, two more effects conspire against the humble craton. Firstly, the velocity of mantle convection increases - this inevitably leads to a higher stress environment for cratons. Secondly, since the phase change at 670 km is endothermic, the layering is enhanced under hotter mantle conditions, and avalanches are more violent and spectacular. But a third mechanism, previously unrecognised, acts to save cratons from these destructive effects. Mantle viscosities are highly sensitive to temperature, and under hotter mantle conditions in the past, the drop in bulk mantle viscosities would be dramatic. This acts to minimise the stresses transferred to the surface plates, and thus to cratons. The modelling shows that the magnitude of this effect far outweighs the stress increase due to faster convection, and craton stability for billions of years is not paradoxical at all in these simulations, for reasonable cratonic properties (see GEMOC Publication #479).
Figure 2. A stable craton with intrinsically strong roots resists tectonically violent mantle avalanches and wildly fluctuating regional stress, in part due to its physical properties, but also due to mild stresses of the ‘warm bath’ Archean mantle environment.
Contacts: Craig O’Neill, Sue O'Reilly, Bill Griffin
Funded by: Macquarie University Research Fellowship